Last year the U.S. Nuclear Regulatory Commission issued a construction and operating license to General Electric-Hitachi Global Laser Enrichment LLC for its proposed uranium enrichment plant in North Carolina, which will use the Separation of Isotopes by Laser Excitation (SILEX) process to enrich uranium for commercial nuclear reactor fuel. Today, the majority of U.S. enriched uranium is supplied by foreign sources; tomorrow the GLE facility promises to secure domestic enrichment services for the next generation of reactors.
On Sept. 25, 2012, the U.S. Nuclear Regulatory Commission (NRC) issued a construction and operating license (COL) to General Electric-Hitachi Global Laser Enrichment LLC (GLE) for its uranium enrichment plant using the Separation of Isotopes by Laser Excitation (SILEX) process. This third-generation process uses lasers to separate or enrich UF6 gas—the working medium of the enrichment process—in uranium-235 (U-235). Once the plant is operational, it will sell enrichment services to clients (including the U.S. government) to produce low-enriched uranium fuel for nuclear reactors.
The Need for Enrichment Services
According to the U.S. Energy Information Administration, the demand for enriched uranium in the U.S. is being supplied by three sources:
■ Domestic production: 16% of U.S. demand.
■ The Megatons-to-Megawatts Program: about 37% of U.S. demand.
■ Other foreign sources: about 47% of U.S. demand. Countries that produce and export enriched uranium to the U.S. include China, France, Germany, the Netherlands, and the United Kingdom.
The primary U.S. uranium enrichment facility is the Paducah Gaseous Diffusion Plant (GDP) in Paducah, Ky., operated by USEC Inc.’s subsidiary, the United States Enrichment Corp. (USEC). The Paducah GDP is scheduled to be de-leased back to the Department of Energy in 2013. This expected closure will decrease domestic production capability.
USEC’s former Portsmouth GDP in Piketon, Ohio, ceased production in May 2001, and parts of that site are undergoing decommissioning, while the Oak Ridge site, K-25, ceased operation in 1987 and is undergoing decommissioning and demolition. The current 16% domestic production is in sharp contrast to the 1970s, when these plants provided more than 90% of the enriched uranium for the western world’s nuclear plants. An August 2012 web posting by URENCO USA in Eunice, N.M., notes that company has been in commercial operation since June 2010 and that “Construction of the project will continue until the plant reaches the planned 5,700 tSW/a [metric tons of separated work per year] capacity.” URENCO USA, the first enrichment facility to be built in the U.S. in 30 years, uses centrifuge enrichment technology and has contracts with around 50 utilities in 17 countries.
The second source, the Megatons-to-Megawatts program, implements a 1993 government-to-government agreement between the U.S. and Russia that calls for Russia to convert 550 U.S. tons of highly enriched uranium from dismantled nuclear warheads into low-enriched uranium (LEU). This is equivalent to about 20,000 nuclear warheads. USEC purchases the enriched portion of the “downblended” material, tests it to verify that it meets specifications, adjusts the enrichment level if needed, and then sells it to its electric utility customers for fuel in commercial nuclear reactors. All program activities in the U.S. take place at the Paducah GDP.
Despite the scheduled ending of the Megatons-to-Megawatts Program in 2013, USEC Inc. signed an agreement in March 2011 with Russian corporation JSC “Techsnabexport” (TENEX) to import, under a new 10-year agreement, LEU from Russia at levels initially expected to reach approximately one-half the level of the Russian downblended weapons-grade materials in 2015. The agreement includes an option to increase quantities equivalent to the Megatons-to-Megawatts Program.
The current five-year average U.S. demand for enriched uranium is approximately 14 million separative work units (SWU) per year, and could reach 15 to 16 million SWU by 2025, depending on the rate of nuclear generation growth in the U.S. (“Separative work unit” describes the effort needed to separate uranium-235 and uranium-238 atoms in natural uranium to create a final product that is enriched in uranium-235 atoms. It takes about 100,000 SWU of enriched uranium to fuel a typical 1,000-MW commercial nuclear reactor for a year.) The overwhelming percentage of domestic enrichment capacity being provided by foreign sources imposes obvious reliability risks, and potential detrimental impacts to national energy security.
To help fill the anticipated supply deficit, other potential domestic sources have emerged in recent years. For example, the NRC issued licenses to USEC Inc.’s American Centrifuge Plant (ACP) in Piketon, Ohio, in 2007 and AREVA Enrichment Services LLC’s Eagle Rock Enrichment Facility (EREF) in Bonneville County, Idaho, in 2011. These facilities are based on the gaseous centrifuge technology. According to the NRC, when the ACP was licensed, it was expected to produce 3.5 million SWU annually, with peak capacity of 3.8 million SWU. The EREF is licensed to produce up to 6.6 million SWU annually.
Three Generations of Enrichment Technologies
Of the processes invented for enriching uranium, only three—gaseous diffusion (first generation), gas centrifuge (second generation), and laser excitation (third generation)—have been considered for commercial use. Only the gaseous diffusion and gas centrifuge technologies have been deployed for large-scale industrial applications. In the case of laser enrichment (which many countries have attempted), Atomic Vapor Laser Isotope Separation (AVLIS) and Molecular Laser Isotope Separation (MLIS) processes (discussed below) have been the most notable, though both have been superseded by SILEX. Two other methods, Aerodynamic Isotope Separation and Chemical and Ion Exchange Enrichment, were never developed on an industrial scale due to a variety of factors such as cost and excessive power requirements.
In the early 1970s, significant work began on the development of laser isotope separation technologies for uranium enrichment. Funding of about $2 billion would be invested to develop the technology at Lawrence Livermore National Laboratory and to successfully demonstrate it with an integrated, full-scale pilot plant. The AVLIS process derived from that work (Figure 1) is based on the circumstance that different isotopes of the same element, though chemically identical, have different electronic energies and absorb different colors of laser light.
| 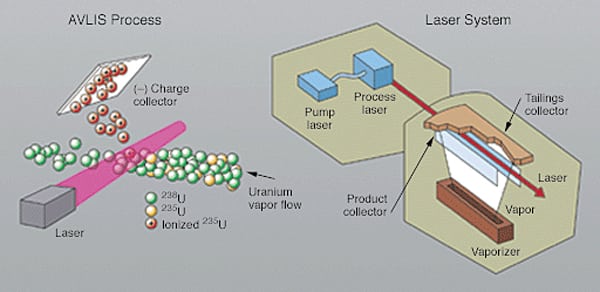 |
---|
1 | 1. One laser enrichment process. In the laser system used for uranium enrichment (right), electrons from U-235 atoms are separated (left), leaving positively charged U-235 ions that can be easily collected for use. This Atomic Vapor Laser Isotope Separation (AVLIS) process has been superseded by the SILEX process. Source: Lawrence Livermore National Laboratory |
---|
The isotopes of most elements can be separated by a laser-based process, if they can be efficiently vaporized into individual atoms or molecules. In AVLIS, uranium metal is vaporized, and the vapor stream is illuminated with a laser light of a specific wavelength that is absorbed only by uranium-235. The laser selectively adds enough energy to ionize or remove an electron from U-235 atoms while leaving the other isotopes unaffected. The ionized U-235 atoms are then collected on negatively charged surfaces inside the separator unit. The collected material (enriched product) is condensed as a liquid on the charged surfaces and then drains to a caster, where it solidifies as metal nuggets. The high separation factor in AVLIS requires fewer stages to achieve a given enrichment, requires less energy, and results in smaller waste volume compared with gaseous diffusion and centrifuge technologies. In 1985, the U.S. Government identified AVLIS as a potential replacement for the gaseous-diffusion technology, but work using this approach was discontinued in 1999 (which was also the year the U.S. signed a technology transfer agreement for the SILEX process).
Like AVLIS, the MLIS process uses a tuned laser to excite U-235 molecules in the UF6 feed gas. A second laser then dissociates excited molecules into UF5 and free fluorine atoms. The enriched UF5 then precipitates and is filtered as a powder from the feed gas. Each stage of enrichment requires conversion of enriched UF5 back to UF6. The advantages of MLIS include low power consumption and the use of UF6 as a process gas. However, it is less efficient and up to four times more energy intensive than AVLIS.
Laser separation is not restricted to a maximum separation factor. In theory, the laser can selectively excite U-235 atoms from the gas mixture and achieve single-stage enrichment. However, what is still unknown (and what is known is classified) is the practical performance of the laser-based stage, both in enrichment factor (will it need a cascade of stages?) and in capacity (how many cascades must be operated in parallel?).
Both of these early efforts at laser separation have now been supplanted by the SILEX process.
SILEX Licensing History
The classified SILEX technology was developed by Australian scientists Dr. Michael Goldsworthy and Dr. Horst Struve in the 1990s, working for Silex Systems Ltd., a public company listed on the Australian Stock Exchange. Technical details of the laser-based enrichment technology are proprietary, are subject to export controls, and, in many cases, may also fall into the categories of security-related, safeguards, or classified information, to which access is limited by Australian and U.S. laws and regulations (Figure 2).
| 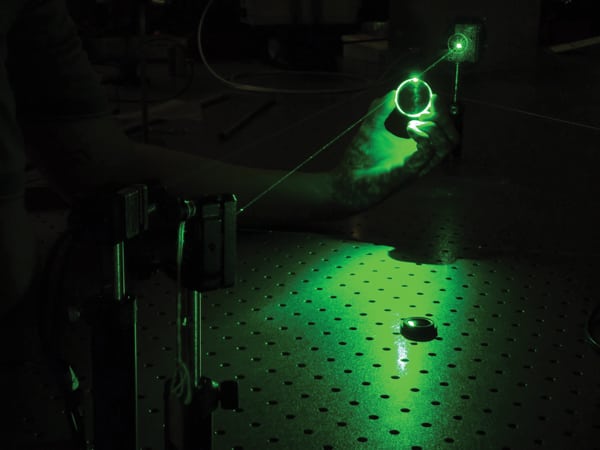 |
---|
1 | 2. Secret process. Many details of the SILEX process are proprietary and classified. This “green laser” image provided by the licensing company is more suggestive than revealing. Courtesy: Silex Systems Ltd. |
---|
The SILEX technology was transferred from Silex’s laboratories in Sydney to the U.S. in 2007 under the “Agreement for Cooperation between the United States of America and Australia Concerning Technology for the Separation of Isotopes of Uranium by Laser Excitation,” signed on Oct. 28, 1999. The technology is being commercialized by GLE under a “Technology License and Commercialization Agreement” originally signed between Silex and GE in 2006. This recently updated agreement provides for a phased commercialization program for the technology (see sidebar “The Path Forward”), with milestone payments to Silex including a $15 million payment announced in May 2013 for completing the Phase 1 Test Loop Program, signaling successful technology validation. The agreement ultimately provides Silex with a very lucrative revenue royalty of between 7% and 12%, depending on the capex spend per unit production capacity.
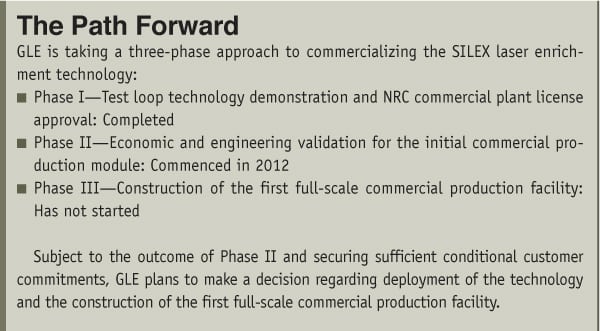
GE brought in its Japanese nuclear partner Hitachi in 2007 and formed GLE, and in 2008 it added Cameco Corp., one of the world’s largest uranium producers. GLE is currently funded by these three parent companies—GE (51% ownership), Hitachi (25%), and Cameco (24%)—through the second phase of the program, which aims to establish engineering and economic validation for a full-scale production plant. GE control of GLE ensures that only Governing Board members who are U.S. citizens with appropriate U.S. government clearances have access to, or exercise control over, activities affecting the protection of the project’s classified information.
GLE submitted its license application on June 26, 2009. The NRC staff conducted thorough safety and environmental reviews of the proposed facility. A safety evaluation report (SER) and final environmental impact statement (EIS) were published in February 2012. The SER documented the staff’s conclusions that GLE’s proposed facility in Wilmington, N.C., complies with NRC regulations and would not pose an undue risk to the health and safety of workers or the public. The EIS concluded there would be no significant environmental impacts that would preclude licensing the facility.
The NRC’s review of the GLE license application provided several opportunities for public comment and participation, including public meetings in Wilmington in July 2008. A notice of opportunity to request an adjudicatory hearing was published Jan. 13, 2010; however, no hearing requests were received. Under NRC regulations, the Atomic Safety and Licensing Board conducted a mandatory hearing on the staff’s review in July 2012, and the Board issued its decision on Sept. 19 authorizing NRC staff to issue the license.
The SILEX Process
Natural uranium ore consists of 99.3% U-238, 0.72% U-235, and trace amounts of U-234 (0.0055%). In order to be used as nuclear fuel in the U.S., U-235 must be increased to 3% to 5% by weight. Enrichment increases the percentage of the naturally occurring and fissile U-235 and decreases the percentage of U-238. The SILEX process consists of four major processing steps and two enrichment support systems to enrich the natural UF6 up to 8% in U-235.
The major enrichment processing steps take place in the following areas:
■ UF6 Feed and Vaporization Area: receives feed cylinders containing UF6 in solid form. The Vaporization Area converts the solid form UF6 into a gas and flows the vaporized UF6 to the feed header, from the Vaporization Area to the Cascade/Gas Handling Area within the Operations Building. The UF6 feed rates to the feed header would be maintained within design basis temperature and pressure ranges. The UF6 is now in a gaseous form. Some stubborn UF6 material may remain within the feed cylinders in a formation called a “heel.” Heel removal usually requires applying a vacuum for the UF6 to volatize for removal and processing.
■ Cascade/Gas Handling Area: where the laser-based enrichment process occurs. The process utilizes lasers tuned to specific frequencies to selectively excite the U-235 feed portion of the UF6 gas molecules, enabling a type of magnetic separation of the U-235 atom from the UF6 feed stock. The result is two trains of materials: the enriched UF6 (product) and depleted UF6 (tails). The enriched stream is subjected to multiple cycles, called a cascade process, until it reaches the desired enrichment level, where it is collected in the Product Withdrawal Area. The depleted stream is sent to and collected in the Tails Withdrawal Area. The feed, product, and tails streams are all in the form of gaseous UF6. The product stream is recovered and stored, in a solid form, in cylinders that meet U.S. Department of Transportation (DOT) offsite cylinder shipping requirements.
■ Product Withdrawal Area: empty to-be-filled cylinders (typically B30 cylinders) are received from interim storage and filled with the enriched UF6 product.
■ Tails Withdrawal Area: receives empty cylinders from interim storage and fills them with the tails (depleted UF6) for interim storage and later disposition.
The two enrichment support systems are:
■ Blending Area: Here the product cylinders that meet the customer specifications would be filled. This is accomplished by mixing the right quantities (by weight) of enriched product of different enrichment levels to produce the exact enrichment level required by customers. The 30- or 48-inch cylinders containing the original product (called donor cylinders) would be received from interim storage. The UF6 from the donor cylinders would be vaporized, blended to the customer’s enrichment specification, and then stored in receiver cylinders that are sampled (in the next step) to confirm final enrichments before they are sent to the customer.
■ Sampling: In this stage the receiver cylinders are sampled to ensure that the level of enriched UF6 in filled cylinders meets customer specifications. To do this, the cylinders are heated to liquefy the UF6 (similar to a boiling process) until the cylinder’s contents are uniformly blended before sampling. This is the only location in the facility where UF6 is converted into its liquid phase.
There are three UF6 cylinder pads:
■ The Product Pad, occupying 48,000 square feet for storing enriched product in 30-inch cylinders.
■ The In-Process Pad, occupying 130,000 square feet for storing feed material and cylinders emptied on site (with or without heels).
■ The Tails Pad, occupying 465,000 square feet for storing 48-inch cylinders containing tails (depleted UF6). This pad would be sized to accommodate the tails cylinders resulting from 10 years of facility operation (9,000 cylinders).
Plant Capacity
The SILEX facility will process up to 154,000 tons of source material in forms of depleted, natural, and enriched uranium. The facility will have a nominal capacity of 6 million SWUs. It is expected that quantities of technetium-99 (Tc-99) and transuranics will be present from residual contamination as a consequence of the historical feed of recycled uranium at other facilities, but these are expected to have no significant impact on operations.
Following startup, peak production is expected to be achieved in 2020. Prior to license expiration in 2052, GLE would decide whether or not to renew its operating license or decontaminate and decommission the facility.
Safety Analysis
GLE plans to construct the plant at the site of GE-Hitachi’s existing Global Nuclear Fuel-America’s fuel fabrication plant in Wilmington, N.C., on 100 acres of an existing 1,621-acre site where GE fabricates commercial nuclear reactor fuel, services reactor control rod drive assemblies, fabricates nuclear reactor components, and builds aircraft engines. This site is located 6 miles north of Wilmington. Construction activities are projected to take place over a seven- to eight-year period and generate more than 3,800 jobs, yielding $139.8 million in income, including $1.7 million in state income taxes and $1.2 million in state sales taxes.
NRC staff will conduct inspections during construction and operation of the GLE facility. The agency plans to hold a public meeting in Wilmington before construction is scheduled to begin to explain its oversight plans to the public.
The facility is licensed under 10 CFR Part 70, Domestic Licensing of Special Nuclear Material, which is applicable to fuel cycle facilities regulated by the NRC. Unlike nuclear reactors, fuel cycle facilities rarely have similar systems and equipment. Because of the uniqueness of these facilities and proprietary processes, safety system requirements at fuel cycle facilities are much less complex than those for reactors, where systems are required to prevent core damage. Nevertheless, safety concerns raised by the 2011 nuclear disaster in Japan have been addressed (see sidebar).
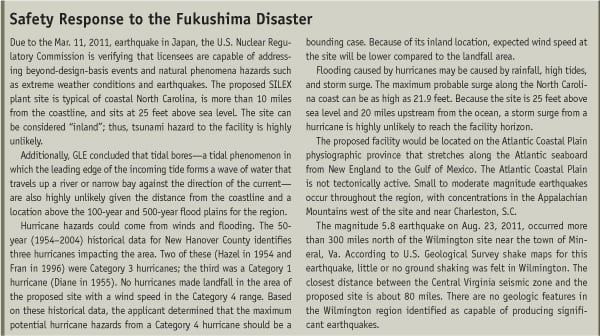
For fuel cycle facilities, instead of a probabilistic risk analysis, affected licensees are required to develop an integrated safety analysis, an established performance-based framework known in the chemical industry as a process hazard analysis. It is used to:
■ Identify initiating events and potential accident sequences from plant operations and external hazards.
■ Identify items relied on for safety (IROFS) such as structures, systems, equipment, components, and activities of personnel that are necessary to prevent these potential accidents and/or mitigate their consequences (such as exceeding radiological or chemical exposure thresholds).
■ Identify the implementation of management measures demonstrating that the IROFS are available and reliable to perform their function when needed.
■ Identify the inclusion of the safety bases.
■ Allow for the licensee to make certain changes to its safety program and facilities without prior NRC approval.
The UF6 process systems in the proposed facility will generally operate at sub-atmospheric pressure. This design feature prevents UF6 leakage. If a process leak were to occur, air in-leakage would initially result, causing a system pressure increase, which may typically be sensed by instrumentation and initiate applicable alarms, system isolation, and enable automated or manual shutdown of impacted systems. Because UF6 reacts with moisture in air, the resultant UO2F2 would be visible and the presence of HF would easily be detected due to its strong odor even below hazardous concentrations. The facility will have IROFS in place and implement defense-in-depth practices as part of facility design.
This ensures that the handling of nuclear material is within the programmatic requirements, that the safety program is appropriate for the risk, and that the measures for carrying out the safety program are appropriately monitored. However, with respect to compliance with the regulations of this part, the NRC requirement is limited to the effects of all relevant hazards on radiological safety, prevention of nuclear criticality accidents, or chemical hazards directly associated with NRC-licensed radioactive materials.
Several organizations have expressed concerns about SILEX technology’s potential to more easily enable nuclear weapons proliferation. The process’s Australian developers say access to the classified process can be safeguarded, and the Australian Department of Foreign Affairs has said that “the SILEX process is technically complex and manufacturing SILEX equipment and components would require very specialised industrial and scientific capabilities, limited to a few countries.” As with any nuclear-related technology, it would appear that the knowledge exists—the genie is out of the bottle—so it is up to responsible parties with access to that knowledge to safeguard it.
A Promising Future
The SILEX process offers certain advantages of operating and capital costs over both gaseous diffusion and gas centrifuge processes. Advancements over earlier laser separation technologies consist of anticipated high separation factors, low energy intensity, low cooling water requirements, a smaller facility footprint, and low capital and operating costs for the same SWU capacity. ■