There may be another player as well perhaps?
https://scitechdaily.com/supersolid-quantum-gases-in-a-new-dimension/
Supersolid Quantum Gases in a New Dimension
TOPICS:Quantum PhysicsSupersolidsUniversity of Innsbruck
By University of Innsbruck August 18, 2021
Two-dimensional supersolid quantum gas produced in the laboratory for the first time. Credit: IQOQI Innsbruck/Harald Ritsch
Two-dimensional supersolid quantum gas produced in the laboratory for the first time.
Quantum gases are very well suited for investigating the microscopic consequences of interactions in matter. Today, scientists can precisely control individual particles in extremely cooled gas clouds in the laboratory, revealing phenomena that cannot be observed in the everyday world. For example, the individual atoms in a Bose-Einstein condensate are completely delocalized. This means that the same atom exists at each point within the condensate at any given time.
Two years ago, the research group led by Francesca Ferlaino from the Department of Experimental Physics at the University of Innsbruck and the Institute of Quantum Optics and Quantum Information at the Austrian Academy of Sciences in Innsbruck managed for the first time to generate supersolid states in ultracold quantum gases of magnetic atoms. The magnetic interaction causes the atoms to self-organize into droplets and arrange themselves in a regular pattern.
“Normally, you would think that each atom would be found in a specific droplet, with no way to get between them,” says Matthew Norcia of Francesca Ferlaino’s team. “However, in the supersolid state, each particle is delocalized across all the droplets, existing simultaneously in each droplet. So basically, you have a system with a series of high-density regions (the droplets) that all share the same delocalized atoms.”
This bizarre formation enables effects such as frictionless flow despite the presence of spatial order (superfluidity).
New dimensions, new effects to explore
Until now, supersolid states in quantum gases have only ever been observed as a string of droplets (along one dimension). “In collaboration with theorists Luis Santos at Leibniz Universität Hannover and Russell Bisset in Innsbruck we have now extended this phenomenon to two dimensions, giving rise to systems with two or more rows of droplets,” explains Matthew Norcia. This is not only a quantitative improvement, but also crucially broadens the research perspectives.
“For example, in a two-dimensional supersolid system, one can study how vortices form in the hole between several adjacent droplets,” he says. “These vortices described in theory have not yet been demonstrated, but they represent an important consequence of superfluidity,” Francesca Ferlaino is already looking into the future. The experiment now reported in the journal Nature creates new opportunities to further investigate the fundamental physics of this fascinating state of matter.
New research field: Supersolids
Predicted 50 years ago, supersolidity with its surprising properties has been investigated extensively in superfluid helium. However, after decades of theoretical and experimental research, a clear proof of supersolidity in this system was still missing. Two years ago, research groups in Pisa, Stuttgart and Innsbruck independently succeeded for the first time in creating so-called supersolids from magnetic atoms in ultracold quantum gases. The basis for the new, growing research field of supersolids is the strong polarity of magnetic atoms, whose interaction characteristics enable the creation of this paradoxical quantum mechanical state of matter in the laboratory.
Reference: “Two-dimensional supersolidity in a dipolar quantum gas” 18 August 2021, Nature.
DOI: 10.1038/s41586-021-03725-7
https://patents.google.com/patent/US20120147906
Abstract
A laser cooling system includes a substrate, an REO layer of single crystal rare earth oxide including at least one rare earth element positioned on the surface of the substrate, and an active layer of single crystal semiconductor material positioned on the REO layer to form a semiconductor-on-insulator (SOI) device. Light guiding structure is at least partially formed by the REO layer so as to introduce energy elements into the REO layer and produce cooling by anti-Stokes fluorescence. The active layer of single crystal semiconductor material is positioned on the REO layer in proximity to the light guiding structure so as to receive the cooling.
Images (7)
Classifications
H01L23/34 Arrangements for cooling, heating, ventilating or temperature compensation ; Temperature sensing arrangements
View 1 more classifications
US20120147906A1
United States
Download PDF
Find Prior Art
Similar
Inventor
David L. Williams
Andrew Clark
Michael Lebby
Current Assignee
IQE PLC
https://patents.google.com/patent/US20120147906
Application US12/966,394 events
2010-12-13
Application filed by Williams David L, Andrew Clark, Michael Lebby
2010-12-13
Priority to US12/966,394
2012-06-14
Publication of US20120147906A1
2014-06-16
Assigned to TRANSLUCENT, INC.
2014-08-05
Application granted
2014-08-05
Publication of US8794010B2
2018-06-07
Assigned to IQE PLC
Status Active
Adjusted expiration 2031-12-23
https://www.electronics-cooling.com/2019/05/whatuse-lasers-and-leds-for-cooling/
What…Use Lasers and LEDs for Cooling?
May 15, 2019 Bill Schweber Articles, Electronics Cooling Blog, Featured, LED / Lighting, Research Laser Cooling
In a recent blog “Some New Cooling Ideas are Needed Here,” I suggested (hoped?) that someone would figure out how to leverage the thermal laws of physics to devise innovative cooling systems which go beyond widely used, conventional techniques of conduction, convection, and radiation. The reason for looking beyond these solutions is that they seem to have reached a limit as to what they can do in practical systems, given the high cooling demands of today’s heat-intensive designs. Whether you use heat sinks, unforced air flow, forced air, liquid cooling, phase change materials, heat pipes, cold plates, or similar well-known embodiments of thermal-management techniques, there is only so much you can do in practice.
But there’s another technique that is less known yet very viable, at least at the atomic scale: the use of lasers for cooling. Perhaps you’re wondering, “Doesn’t the laser’s photon stream actually add energy and thus heat to the target? Isn’t this counterproductive?” The answer is “yes” to the first question, and maybe “no” to the second one. Just because it’s a source of heat doesn’t mean it can’t be used for cooling: think of air conditioners which are powered by a gas line and have an internal heating system. Although it is very counterintuitive to the non-technical layman, that arrangement can be used to create cooling via a no-compressor absorption process (Reference 1).
Although laser-based cooling may seem bizarre at first, it is both practical and even available as an off-the-shelf system. It’s based on deep understanding of quantum physics, and the physics supporting this counterintuitive technique is actually well established and is based on the work of physicists such as Arthur Ashkin (who shared the Nobel Prize in Physics in 2018 for his work on optical tweezers) and many others.
Laser cooling is actually a broad term which includes several techniques in which atomic and molecular samples are cooled down to near absolute zero. In very simple terms, it uses the fact that when an object (usually an atom) absorbs and re-emits a photon, its momentum changes, Figure 1. If there is a group (ensemble) of particles, their temperature will be proportional to the variance in their velocity, with more homogeneous velocities among particles corresponding to a lower temperature. The laser beam initiates cooling by “compressing” the velocity distribution of the particle ensemble, which results in cooling the particles.
Fig 1: In laser Doppler cooling, the atoms to be cooled are surrounded (“trapped”by lasers which are tuned to a frequency slightly below an electronic transition in the atom. If an atom is moving in the direction of one of the laser beams, the light will be blue-shifted to where the atom can absorb the light. Then atom’s motion will be slowed, and the excited atom spontaneously emits a photon. As theses emissions are in random direction, so that on average, the momentum of the atom ensemble – and thus its temperature – are reduced. (Image source: Quora)
Despite its esoteric nature or “magic-like” aspects (depending on your perspective), laser-based cooling is not just a laboratory curiosity. It is already used for off-the-shelf, vibration-free cryogenic cooling devices used for precision optical instrumentation (where any hint of vibrating is unacceptable) as well as exploration of states of matter such as Bose–Einstein condensates. The most-common variation of laser cooling is called Doppler cooling (see References 1, 2, and 3 for a high-level explanation, a mid-range one, and an equation-intense academic one, respectively) and has been used, for example, to cool rubidium-85 atoms down to several hundred microK and below by trapping the target with multiple laser beams – that’s very close to absolute zero.
But multi-lasers traps are complicated, and trapping atoms and molecules is definitely challenging. Still, the idea of using photon emission and absorption for cooling is attractive, so much so that researchers at the University of Michigan (Ann Arbor, MI) have explored use of a standard infrared light-emitting diode (IR LED) – but connected with its electrodes reversed – to cool another device just nanometers away (no, this is NOT a joke!).
In their arrangement, when the LED was reverse biased, it began acting as a very low-temperature object and absorbed photons (representing energy) from the custom calorimeter which was positioned nearby using a piezo-based actuator, Figure 2. A subwavelength gap of a few tens of nanometers between the LED and the calorimeter established what is called near-field spacing and evanescent coupling.
Fig 2: Measuring the radiative conductance: a) schematic of the set-up for measuring radiative conductance GNFRHT. The emitting area of the photodiode is located below the n-InAs layer; b) effective thermal resistance network showing the major heat transfer pathways, where the calorimeter has a power input (Qcal) due to a current supplied to the platinum resistor; c) and d) simultaneously recorded displacement and calorimeter temperature change (∆Tcal) during a GNFRHT measurement. (Image source: University of Michigan)
This cooling occurs via two mechanisms: photon tunneling (which enhances the transport of photons across nanoscale gaps), and suppression of photon emission from the photodiode (due to a change in the chemical potential of the photons under an applied reverse bias). Their work is detailed in their paper published in Nature, “Near-field photonic cooling through control of the chemical potential of photons.”
Who knows into what this may evolve? While this approach is presently suitable only for atomic-level cooling, it does demonstrate that active nanophotonic cooling using IR LEDS in place of coherent laser radiation is feasible. Perhaps it will somehow be expanded and “scaled up” to become the foundation of solid-state, on-chip device cooling based on nanoscale photonics and optoelectronics. As the old saying goes, “never say never!”
Laser cooling is primarily used to create ultracold atoms for experiments in quantum physics. These experiments are performed near absolute zero where unique quantum effects such as Bose–Einstein condensation can be observed.
- Forums
- ASX - By Stock
- SLX
- Zero Spin Silicon
SLX
silex systems limited
Add to My Watchlist
2.33%
!
$3.95
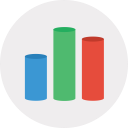
Zero Spin Silicon, page-93
Featured News
Add to My Watchlist
What is My Watchlist?
A personalised tool to help users track selected stocks. Delivering real-time notifications on price updates, announcements, and performance stats on each to help make informed investment decisions.
|
|||||
Last
$3.95 |
Change
0.090(2.33%) |
Mkt cap ! $947.6M |
Open | High | Low | Value | Volume |
$3.91 | $4.01 | $3.88 | $859.5K | 216.2K |
Buyers (Bids)
No. | Vol. | Price($) |
---|---|---|
5 | 1464 | $3.95 |
Sellers (Offers)
Price($) | Vol. | No. |
---|---|---|
$3.96 | 2336 | 10 |
View Market Depth
No. | Vol. | Price($) |
---|---|---|
3 | 72 | 3.970 |
28 | 7269 | 3.960 |
14 | 6120 | 3.950 |
14 | 8253 | 3.940 |
9 | 4449 | 3.930 |
Price($) | Vol. | No. |
---|---|---|
3.980 | 5607 | 19 |
3.990 | 9007 | 14 |
4.000 | 13938 | 18 |
4.010 | 10125 | 11 |
4.020 | 4416 | 6 |
Last trade - 11.56am 10/07/2025 (20 minute delay) ? |
Featured News
SLX (ASX) Chart |